Afdeling
Cel- en Chemische Biologie (Moleculaire celbiologie)
Onze afdeling is gehuisvest in het onderzoeksgebouw en is nauw verwant aan de oudste universiteit van Nederland: de Leidse universiteit. Op de afdeling werken ongeveer 200 onderzoekers, van bachelor- tot master- en PhD-studenten, postdocs en senior wetenschappers. Ze zijn verdeeld over 20 groepen, die onder leiding staan van hoofdonderzoekers. Elk met hun eigen expertise.
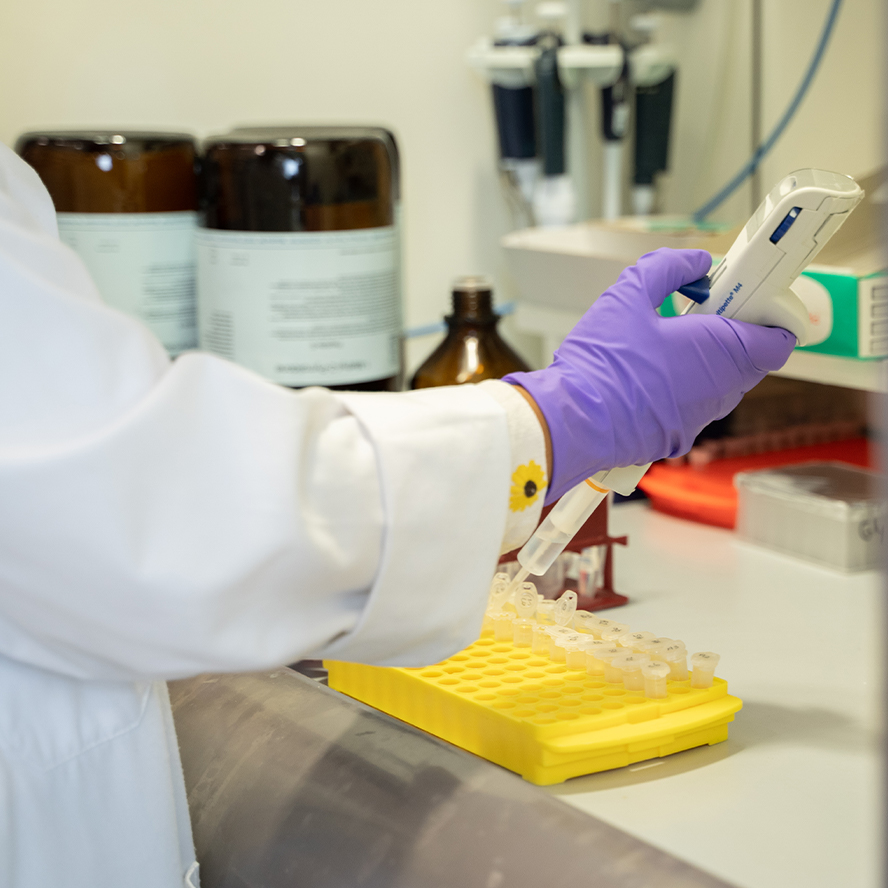&width=710&height=710)
Wat doet onze afdeling?
Over de afdeling
Contactgegevens
For general inquiries, contact our secretarial office:
Pauline Hoftijzer
Management Assistant Cell and Chemical Biology (Neefjes lab, Ovaa lab)
T: +31 (0) 71 526 87 27
E: p.hoftyzer@lumc.nl
F: +31 71 526 8270